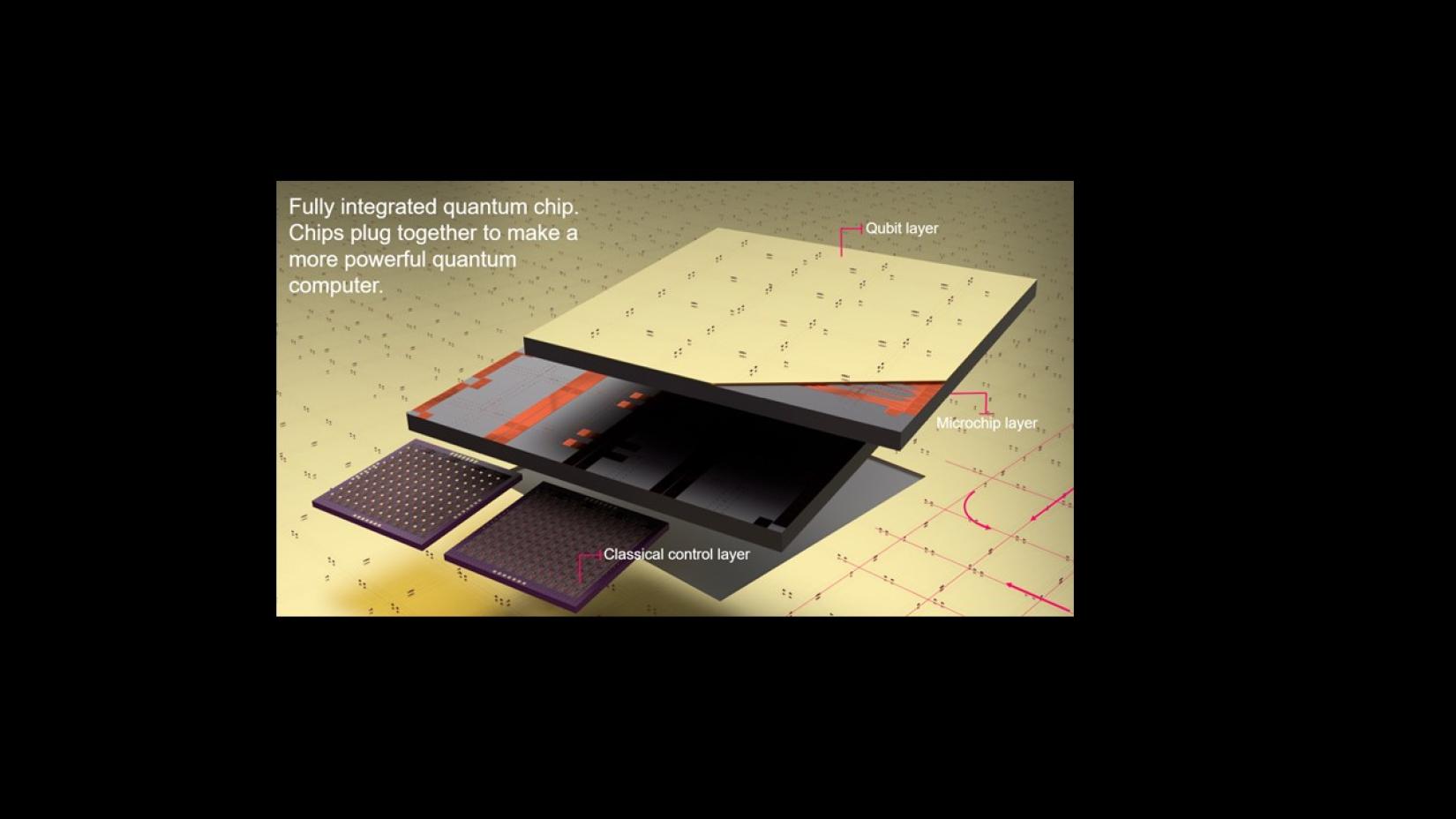
Visual illustration above of the iQPU technology
The ion trap is at the heart of the iQPU, enabling quantum gates and allowing ions to move around [1]. First, let’s explore our gate scheme, which is built for scalability from the ground up. Let’s begin with an analogy: Imagine sitting in your car and turning on your radio to a variety of radio channels you can listen to. As a listener, you can locally tune into one channel and ignore all the others. A nice feature here is that no matter how many listeners there are, the number of radio channels remains constant. Turns out that radio broadcasting provides a useful analogy for a highly scalable quantum gate system.
We apply the same principle with quantum gates, taking advantage of qubits we can tune locally [2]. We use frequencies in the microwave regime to set up channels that we broadcast over all our qubits. Each channel corresponds to a different type of gate, such as a two-qubit gate or a particular single qubit gate. By locally changing the magnetic field our special qubit resides in, we alter the frequency that can interact with it, effectively tuning it into a chosen gate channel, which determines the quantum gate that operates on it. A neat trick we use to locally tune the magnetic field is to engineer a magnetic field gradient that has different magnetic fields at various positions. By simply moving a qubit to a specific location by applying a voltage to the ion trap, we allow it to perform a gate. Since we can move qubits individually, we have scalable control over which qubits perform which gates, whether they are single-qubit gates, two-qubit gates, or doing nothing at all! Like the radio example, whether you have 5 qubits or 5 million, we broadcast the same number of microwave channels, giving us scalable gate technology.
To move ions, we use the quantum charged coupled device architecture [3], [4], which applies voltages to electrodes to move ions around a grid surface—like a game of atomic Pac-Man. In different grid sections, various operations, such as quantum gates or state readout, can occur. What’s particularly cool is that we can reliably move one qubit to another, giving us all-to-all connectivity. This capability allows us to implement more efficient quantum error correction codes, drastically reducing the overhead of qubits needed for error correction [6]. To enable such an architecture one requires a complex microfabricated ion trap chip. However, when considering large-scale systems, the required level of control introduces a challenge—the so-called “tyranny of numbers.”
If you visit your local ion trap lab, you’ll witness this “tyranny” in the nests of wires. To control a single qubit, you need several wires to apply signals. Worse still, doubling the qubits means doubling the wires. Once you start considering thousands of qubits, scalability becomes an issue. Fortunately, this problem has been well explored in the classical world and is known as Rent’s Rule, which states that you cannot indefinitely double the wires needed to control a device's increased power. This has been extended to quantum computers in a superb paper by Franke et al. [7]. At Universal Quantum, we solve this problem with active control via an Application Specific Integrated Circuit (ASIC). This classical chip, made in an established CMOS fabrication facility, changes the game in terms of how the wires scale. Crucially, we integrate this ASIC together with our ion trap chips to form the integrated quantum processing unit (iQPU).
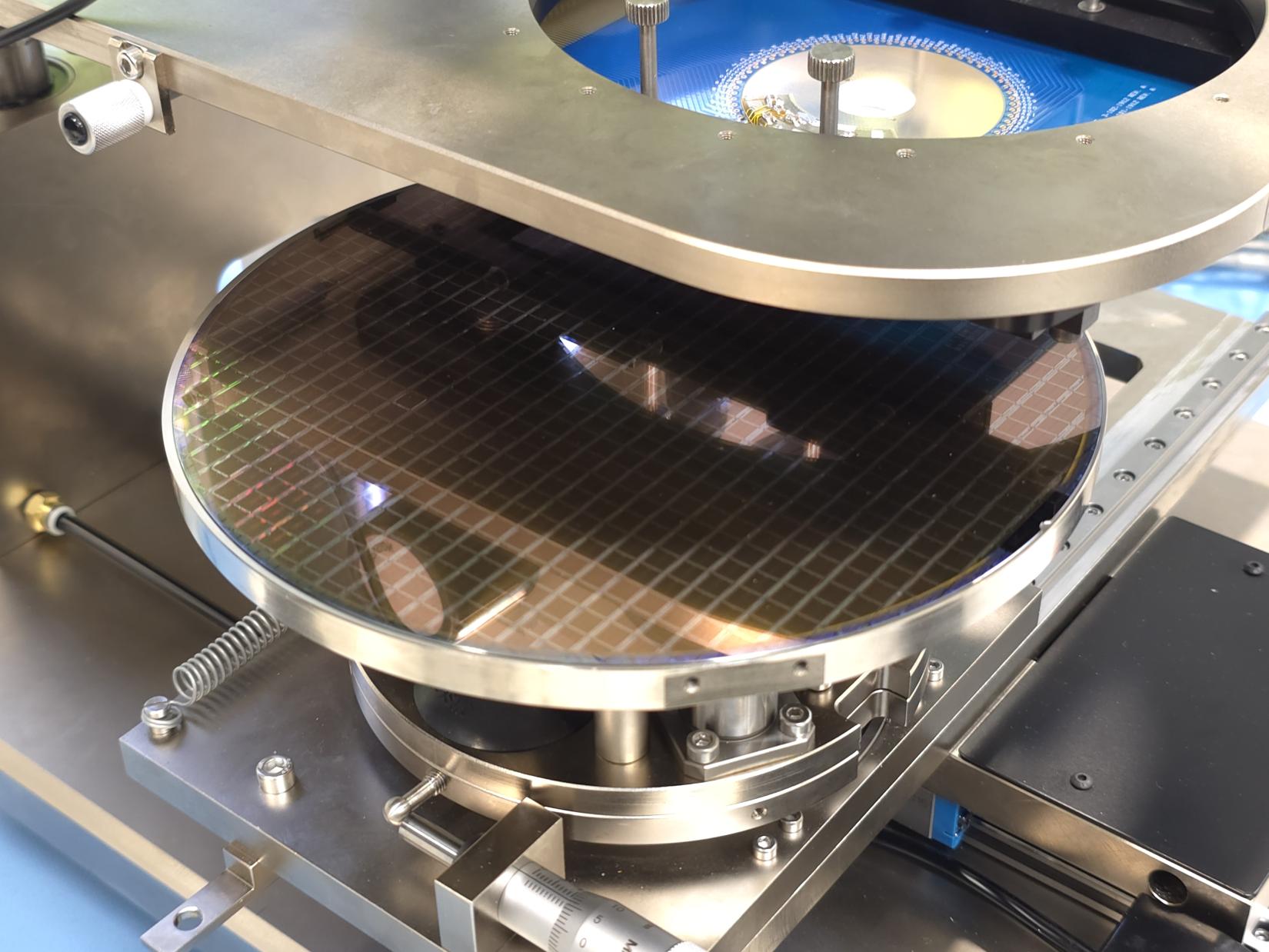
Image above of UQ's recently produced ASIC technology
“So, you’ve proposed an iQPU—can it scale?”
One quantum module or iQPU can hold only so many qubits, and solving real problems will require a lot of them [8]. As a result, we need a way to connect our iQPUs. Connecting quantum computing modules is seen as one of the biggest challenges for most quantum computing architectures. At Universal Quantum, we’ve taken a unique approach to this challenge. We use UQConnect, which engineers a special interface to connect iQPUs so they appear to qubits as one iQPU. This enables us to directly transport qubits between them. This approach achieves world-record module connectivity of over 2000 transfers per second with very high reliability (99.999993%) [9].
“This sounds very complicated to manufacture at scale—can you do it?”
Excellent question! It’s almost as if it’s staged. One of the things I love to say about Universal Quantum is how lazy we are. Why try to invent new fabrication processes when one of the world's largest industries is already dedicated to making chips? Can’t we just piggyback on them? The answer is a resounding “Yes!” We have managed to design our chips to fit into these existing semiconductor processes, which are already manufacturable, reliable, and can be mass-produced. This means we have already transitioned to our chips being produced by standard semiconductor fabrication houses instead of less reliable multi-purpose cleanroom facilities. The key to this is heterogeneous integration—or, as I like to call it, the best of all worlds.
The issue we often face is that the process best suited for making an ASIC isn’t always ideal for making an ion trap. Why not use both? With the magic of advanced packaging, we can tightly integrate ASIC chips made using their specialist process with ion trap chips made using a different, specialized process. Even better, this improves yields. When a single, complex process is used, yields drop due to more points of failure. But by using two simpler processes and testing them before integration, the total yield of the combined unit is higher than doing everything in one process. These tactics enable us to develop complex iQPUs piece by piece, test them, and then integrate them using simpler, standardized chips.
I hope this blog post has demystified some of what’s going on here at Universal Quantum and our unique approach to solving the scalability challenge. Stay tuned for more educational blog posts from the UQ team. Also visit our tech page for more insight into our unique approach here at UQ.
References
[1] B. Lekitsch et al., “Blueprint for a microwave trapped ion quantum computer,” Sci. Adv., vol. 3, no. 2, p. e1601540, Feb. 2017.
[2] S. Weidt et al., “Trapped-ion quantum logic with global radiation fields,” Phys. Rev. Lett., vol. 117, no. 22, pp. 1–6, Mar. 2016.
[3] D. J. Wineland, C. Monroe, W. M. Itano, D. Leibfried, B. E. King, and D. M. Meekhof, “Experimental Issues in Coherent Quantum-State Manipulation of Trapped Atomic Ions,” J. Res. Natl. Inst. Stand. Technol. [J. Res. Natl. Inst. Stand. Technol, vol. 103, no. 103, pp. 1689–1699, 1998.
[4] D. Kielpinski, C. Monroe, and D. J. Wineland, “Architecture for a large-scale ion-trap quantum computer,” Nature, vol. 417, no. 6890, pp. 709–711, Jun. 2002.
[5] P. Kaufmann, T. F. Gloger, D. Kaufmann, M. Johanning, and C. Wunderlich, “High-Fidelity Preservation of Quantum Information during Trapped-Ion Transport,” Phys. Rev. Lett., vol. 120, no. 1, p. 010501, Jan. 2018.
[6] N. P. Breuckmann and J. N. Eberhardt, “Quantum Low-Density Parity-Check Codes,” PRX Quantum, vol. 2, no. 4, p. 1, 2021.
[7] D. P. Franke, J. S. Clarke, L. M. K. Vandersypen, and M. Veldhorst, “Rent’s rule and extensibility in quantum computing,” Microprocess. Microsyst., vol. 67, pp. 1–7, Jun. 2019.
[8] M. Webber, V. Elfving, S. Weidt, and W. K. Hensinger, “The impact of hardware specifications on reaching quantum advantage in the fault tolerant regime,” AVS Quantum Sci., vol. 4, no. 1, p. 013801, Mar. 2022.
[9] M. Akhtar et al., “A high-fidelity quantum matter-link between ion-trap microchip modules,” Nat. Commun., vol. 14, no. 1, p. 531, Feb. 2023.